Table of Contents
- Preventive Cancer Vaccines:
- Therapeutic Cancer Vaccines:
- The Spectrum of Cancer Vaccine Strategies
- Cell-based cancer vaccines:
- Viral-based cancer vaccines
- Peptide-based cancer vaccines:
- Nucleic acid-based cancer vaccines:
- Cancer Vaccine Antigens
- Vaccine Combinations with Immune Checkpoint Inhibitors
- Mouse Models in Preclinical Vaccine Development
Since their development in the 18th century, vaccines have been highly effective in preventing diseases caused by viruses and bacteria, saving hundreds of millions of lives globally. Vaccines work by mimicking an infection, stimulating the body’s natural defences to recognise and combat disease-causing microorganisms. These vaccines typically contain weakened, inactivated, or fragmented forms of the pathogens and are most effective when administered in a preventive context—before the individual is exposed to the virus or bacteria
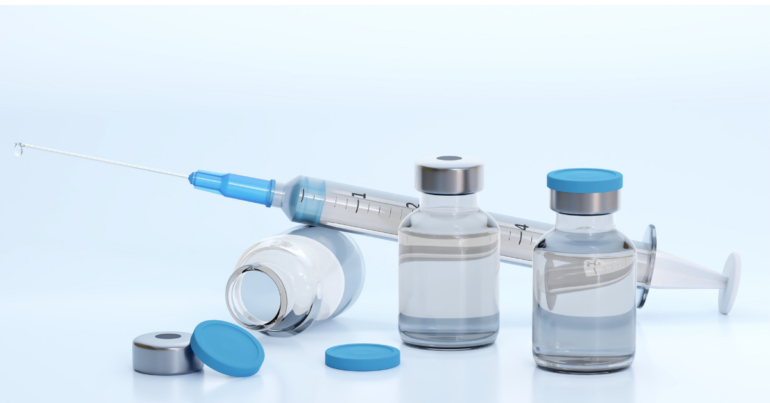
What are Cancer Vaccines?
Cancer vaccines are a type of immunotherapy that leverages the body’s immune system to fight cancer. These vaccines come in two forms: Preventive and Therapeutic.
Preventive Cancer Vaccines:
Some viruses, called oncogenic viruses, can cause or contribute to the development of cancer. Unlike viruses like influenza, which cause acute infections, oncogenic viruses often persist in the body, leading to long-term infections. They can cause cancer by altering cellular genes, suppressing immune function, or inducing chronic inflammation.
Preventive cancer vaccines target these oncogenic viruses, reducing the risk of infection and lowering the chance of developing virus-associated cancers. These vaccines are administered to healthy individuals to prevent cancer before it starts.
Several oncogenic viruses are known to cause cancer, including:
- Epstein-Barr Virus (EBV): Linked to Burkitt’s lymphoma, non-Hodgkin lymphoma, Hodgkin lymphoma, nasopharyngeal carcinoma
- Hepatitis B Virus (HBV): Causes hepatocellular carcinoma
- Hepatitis C Virus (HCV): Linked to hepatocellular carcinoma
- Human Immunodeficiency Virus (HIV): Increases the risk of Kaposi sarcoma, non-Hodgkin lymphoma, cervical cancer
- Human Herpesvirus-8 (HHV-8): Causes Kaposi’s sarcoma
- Human Papillomavirus (HPV): Causes cancers of the cervix, vagina, vulva, penis, anus, and oropharynx
- Human T-Cell Lymphotropic Virus-1 (HTLV-1): Leads to acute T-cell leukemia
- Merkel Cell Polyomavirus (MCV): Causes Merkel cell carcinoma
Among these, preventive vaccines are available for HBV and HPV, with four vaccines approved by the U.S. Food and Drug Administration (FDA):
- Cervarix®: Protects against HPV types 16 and 18, preventing cancers of the anus, cervix, head and neck, penis, vulva, and vagina.
- Gardasil®: Covers HPV types 16, 18, 6, and 11, preventing HPV-related cancers and genital warts.
- Gardasil-9®: Protects against additional HPV types (16, 18, 31, 33, 45, 52, 58) as well as types 6 and 11 for genital warts, helping prevent a wider range of HPV-related cancers.
- HEPLISAV-B®: Prevents hepatitis B infection, reducing the risk of liver cancer.
Therapeutic Cancer Vaccines:
Unlike preventive vaccines, therapeutic cancer vaccines are designed to treat existing cancers. They aim to delay or halt cancer growth, shrink tumours, prevent recurrence, and eliminate residual cancer cells post-treatment. These vaccines stimulate the immune system to specifically target and attack cancer cells.
Therapeutic vaccines are often made from cancer cells, fragments of cells, or cancer-specific proteins, helping the body recognise and combat the disease. In some cases, a patient’s immune cells are collected, exposed to these substances in the lab, and then reinfused to enhance the immune response. Several therapeutic  cancer vaccines have been approved by the FDA and are already in use for different cancers:
- Sipuleucel-T (Provenge®): Used for metastatic castration-resistant prostate cancer, this dendritic cell vaccine involves removing immune cells, stimulating them with PA2024 (a fusion of prostate-specific antigen and prostatic acid phosphatase), and reinfusing them to enhance the body’s ability to fight prostate cancer. Provenge has been shown to extend survival in patients with metastatic prostate cancer.
- Bacillus Calmette-Guérin (BCG): Administered via catheter for early-stage bladder cancer, BCG is a weakened form of mycobacterium that stimulates immune cells to attack bladder cancer.
- Talimogene laherparepvec (T-VEC, Imlygic®): Used for advanced melanoma that can’t be fully removed by surgery, T-VEC is an oncolytic virus-based vaccine made from genetically modified herpes virus to trigger an immune response against cancer.
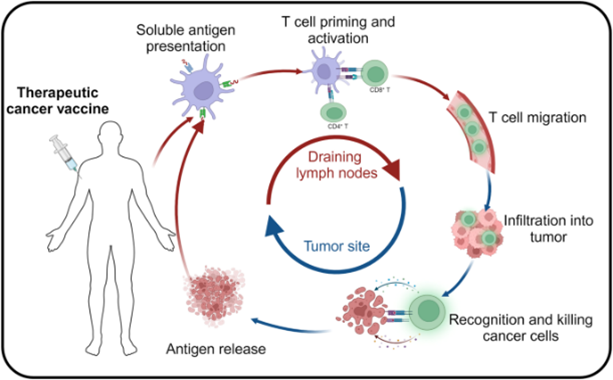
Figure 1: The mechanism of cancer vaccine in vivo. Tumour antigens are phagocytosed and processed by antigen-presenting cells (APCs), which present them on their surface via major histocompatibility complexes (MHC). These MHC complexes activate T-cells by binding to T-cell receptors (TCRs), enabling the targeted and sustained destruction of tumour cells and inhibition of tumour growth.
Credit: Fan T., Zhang M., et. al., doi.org/10.1038/s41392-023-01674-3
Reproduced under the Creative Commons license
The Spectrum of Cancer Vaccine Strategies
The development of cancer vaccines involves diverse strategies to enhance immune recognition of tumour antigens. These approaches vary in how they deliver antigens to trigger a robust immune response. Cancer vaccines can be broadly categorised into cell-based, peptide-based, viral-based, and nucleic acid-based platforms, each offering unique mechanisms to activate the immune system against cancer.
Cell-based cancer vaccines:
Cell-based vaccines can be made from either whole tumour cells (autologous or allogeneic) or dendritic cells (DCs). Autologous tumour vaccines use a patient’s own cancer cells to generate a personalised immune response without needing to identify specific tumour-associated antigens (TAAs) beforehand, but this requires sufficient tumour samples. Allogeneic vaccines, on the other hand, use standardised tumour cell lines for easier production.
Dendritic cells are highly specialised antigen-presenting cells (APCs) that activate naive T cells. Dendritic cell vaccines involve loading a patient’s DCs with peptide antigen or transfected with antigen genes. They have shown promise, particularly in treating metastatic prostate cancer.
Viral-based cancer vaccines
Viral-based vaccines leverage the immune system’s natural ability to respond to viruses, triggering strong and durable immune responses. Vectors like poxviruses, adenoviruses, and herpes simplex virus (HSV) are commonly used, with poxviruses standing out for their ability to carry large transgene inserts. Most viral vectors are either replication-defective or attenuated versions. A key challenge is the body’s immune system neutralising the viral vector, which has led to the development of prime-boost strategies, where a viral vector is followed by a boost using a different vector carrying the same antigen.
Peptide-based cancer vaccines:
Peptide-based vaccines aim to trigger an immune response by targeting TAAs that are either uniquely or overexpressed in cancer cells. Using synthetic peptides, they can be personalised for specific cancers. The peptides are taken up by APCs, presented with HLA molecules, and recognised by T cells, leading to a targeted immune response.
Nucleic acid-based cancer vaccines:
Nucleic acid-based vaccines, including DNA, RNA, introduce genetic material into APCs to produce cancer-specific antigens. Nucleic acid vaccines have demonstrated advantages such as the ability to deliver multiple antigens with a single immunisation, safety, specificity for inducing the immune response for the antigen of interest, induction of both humoral and cellular immune responses, relatively low production cost, and ease of manufacturing. However, DNA vaccines have low cellular uptake efficiency.
RNA vaccines, which primarily use messenger RNA, similarly aim to induce antigen translation in APCs. RNA vaccines are considered safer than DNA vaccines, as RNA degrades more quickly and doesn’t integrate into the genome, reducing the risk of side effects or autoimmune reactions. Additionally, unlike DNA, mRNA vaccines do not need to reach the nucleus as they are translated in the cytoplasm. However, RNA vaccines also face challenges with efficient uptake and antigen production.
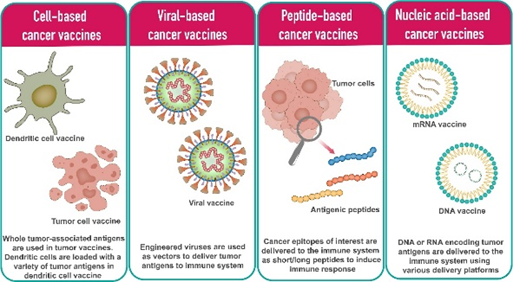
Figure 2: Different types of cancer vaccine platforms.
Credit: Vishweshwaraiah YL. and Dokholyan NV. doi: 10.3389/fimmu.2022.1029069
Reproduced under the Creative Commons license
Cancer Vaccine Antigens
Developing cancer vaccines faces significant challenges due to the nature of tumour antigens. Tumour antigens can be classified into two general classes: (i) tumour-associated antigens (TAAs) that are expressed by the tumour as well as the healthy tissue and (ii) Tumour-specific antigens (TSAs), or neoantigens, for which expression is restricted to the tumour lesion.
Tumor-associated antigens (TAAs) are self-proteins that are overexpressed in malignant cells and classified into three categories: differentiation antigens, widely occurring overexpressed antigens, and cancer testis antigens (CTAs). However, targeting TAAs for cancer vaccines has shown limited success due to their nature as self-antigens. The immune system’s tolerance to self-antigens makes it difficult to elicit a strong immune response against TAAs without risking autoimmunity.
One example is MUC1, a glycoprotein normally expressed on epithelial cells but overexpressed and aberrantly glycosylated in cancers such as breast, lung, and pancreatic cancer. While MUC1-based vaccines have shown promise in triggering immune responses in clinical trials, these responses have often failed to produce significant clinical outcomes like tumour shrinkage or improved survival rates. The weak immunogenicity of self-antigens like MUC1 and tumour evasion mechanisms contribute to the limited success of TAA vaccines.
To improve the efficacy of TAA vaccines, including MUC1, researchers are investigating strategies such as combining vaccines with immune checkpoint inhibitors, using stronger adjuvants, or developing personalised approaches to enhance the immune system’s ability to target and destroy tumour cells.
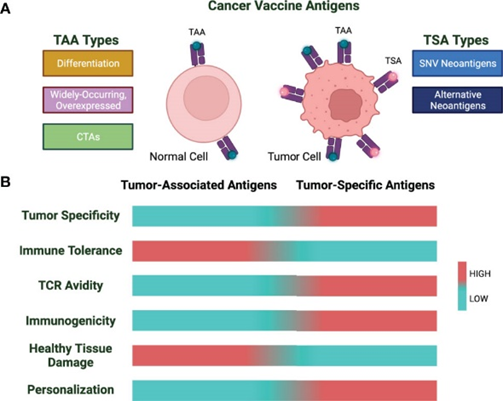
Figure 3 Cancer vaccine antigen types and characteristics. (A) Cancer vaccine antigens are divided into TAA and TSA. (B) TAAs and TSAs are characterized by varying properties.
Credit: Hernandez R. and Malek TR. doi: 10.3389/fonc.2022.878377
Reproduced under the Creative Commons license
Tumour-specific antigens (TSAs), or neoantigens, are unique “non-self” proteins that arise from mutations in cancer cells, such as point mutations, frameshifts, and insertion/deletion events. Tumours with higher mutational burdens produce more neoantigens, making them valuable targets for therapy. A key class of TSAs includes non-synonymous single-nucleotide variant (SNV) neoantigens.
Unlike TAAs, neoantigens have several advantages: they bind more strongly to HLA and T-cell receptors, are exclusively expressed in tumour cells, and avoid issues with central tolerance and autoimmunity. However, only about 1.2% of neoantigens induce a meaningful antitumor response, making precise prediction and validation critical for vaccine development.
Neoantigens can be classified as shared or personalised. Shared neoantigens, found in multiple cancer patients due to common mutations like those in TP53 and KRAS, are suitable for “off-the-shelf” vaccines. Personalised neoantigens, unique to each patient’s tumour, are identified based on individual mutation profiles.
The development of personalised neoantigen-based cancer vaccines relies on advanced technologies like whole-genome and whole-exome sequencing to identify tumour-specific mutations. Bioinformatic algorithms play a crucial role by analysing factors like translation rates, immunological tolerance, and HLA binding affinity to predict which neoantigens could trigger an immune response. Despite many predictions, only a small fraction of neoantigens are truly immunogenic, making validation essential. Tools like mass spectrometry and DNA barcoding help verify neoantigens. Vaccine formulations can include peptides, dendritic cells, or DNA/RNA, depending on the neoantigen’s characteristics.
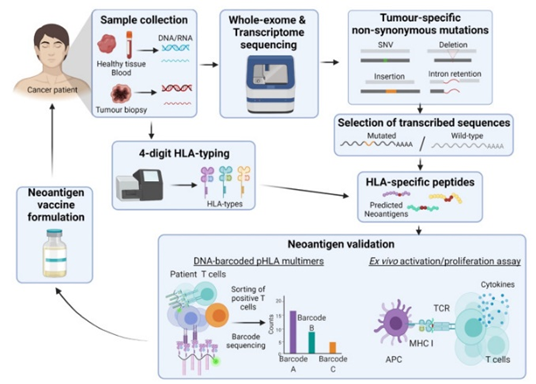
Figure 4 Basic neoantigen vaccine pipeline. From sample collection, whole exosome sequencing, HLA-typing, generation of HLA-specific peptides, neoantigen validation to neoantigen vaccine formulation.
Credit: Tay BQ., Wright Q., et. al. doi: 10.3390/vaccines9050535
Reproduced under the Creative Commons license
Vaccine Combinations with Immune Checkpoint Inhibitors
Pairing cancer vaccines with other immunotherapies is essential for enhancing the effectiveness of cancer treatment. While cancer vaccines are designed to stimulate the immune system to recognise and attack tumour cells, their efficacy can be limited by various factors, such as the tumour’s ability to evade immune detection or insufficient immune activation.
Combining cancer vaccines with immune checkpoint inhibitors (ICIs) is a promising approach to enhance treatment efficacy. Therapeutic cancer vaccines are designed to boost tumour-specific T lymphocytes, which can be further activated by ICIs. While ICIs alone have shown success in cancers like NSCLC and melanoma, their effectiveness in less immunogenic tumours, such as prostate and pancreatic cancers, has been limited. Cancer vaccines help in the activation and localisation of tumour-specific T cells to the tumour microenvironment (TME), and induce antigen spreading through tumour cell death. This process can upregulate immune checkpoint molecules, making combination therapy advantageous.
ICIs work by overcoming the immunosuppressive TME that often limits T-cell function. For instance, CTLA-4 inhibitors can enhance vaccine-induced T-cell responses by counteracting inhibitory signals and shifting the TME from suppressive to permissive. Similarly, PD-1 inhibitors prevent vaccine-activated T cells from becoming senescent, thus sustaining long-term antitumor responses. Overall, combining vaccines with ICIs not only boosts the initial immune response but also helps maintain and strengthen it by addressing the TME’s suppressive nature.
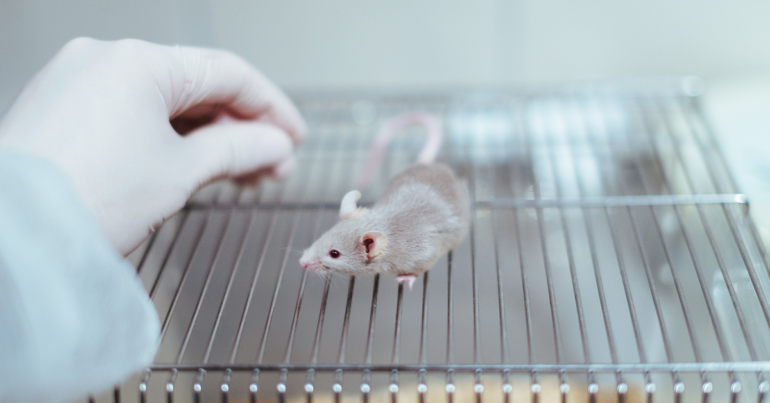
Mouse Models in Preclinical Vaccine Development
When studying cancer vaccines, mouse models are indispensable tools for understanding immune responses and vaccine efficacy. Different types of mouse models are used based on the specific research question. Below are some key models commonly employed in cancer vaccine studies:
1. Syngeneic Mouse Models
Syngeneic models involve transplanting tumour cells from the same genetic background as the host mouse, typically within inbred strains such as C57BL/6 or BALB/c. These models are highly valuable for studying immunotherapy and cancer vaccines because they retain an intact immune system, allowing the investigation of interactions between the tumour, immune responses, and vaccines. Syngeneic models are commonly used to assess vaccine efficacy, immune activation, and therapeutic potential.
Example: The B16 melanoma model in C57BL/6 mice is a widely used syngeneic model for melanoma vaccine studies.
2. Genetically Engineered Mouse Models (GEMMs)
GEMMs are designed to carry specific oncogenic mutations that mimic human cancer development. These models spontaneously develop tumours in the presence of a functional immune system, providing a closer representation of how cancer arises and interacts with the immune system. GEMMs are ideal for studying the effects of cancer vaccines on tumour initiation and progression in an immunocompetent environment. Additionally, GEMMs allow researchers to study tumour antigen expression in a natural setting, making them valuable for exploring neoantigen-based vaccine strategies.
Example: Mice carrying mutations in the KRAS gene can develop tumours mimicking those in humans, allowing for testing of vaccines that target these specific genetic mutations.
3. Xenograft Models
In xenograft models, human tumour cells are implanted into immunodeficient mice, such as the NCG mice. While these models do not have a fully functional immune system, they are widely used to study tumour growth and response to vaccines in combination with other therapies. Xenograft models are particularly useful when the focus is on vaccine efficacy against human tumour antigens. However, the lack of an intact immune system limits their ability to fully mimic the complexities of immune responses, making them less suitable for immunotherapy research compared to syngeneic or humanised models.
Example: Human cancer cells can be implanted into mice that lack T cells (e.g., nude or NCG mice) to observe tumour growth and response to human-targeted therapies.
4. Humanised Mouse Models
Humanised mice are immunodeficient mice reconstituted with human immune cells or tissues, enabling the study of human-specific immune responses. These models are useful for testing cancer vaccines that are designed to stimulate human immune cells, particularly in personalised vaccine approaches. Humanised models allow researchers to explore vaccine-induced responses involving human T cells, dendritic cells, or other immune components. They are especially valuable for preclinical studies when transitioning cancer vaccines from mouse models to human clinical trials.
Example: NCG (NOD scid gamma) mice reconstituted with human peripheral blood mononuclear cells (PBMCs) to study human immune responses to vaccines.
5. Humanised Checkpoint Mouse Models
These mice express human versions of immune checkpoint molecules like PD-1, PD-L1, or CTLA-4. They are particularly valuable for studying cancer vaccines in combination with checkpoint inhibitors, which target these molecules to enhance immune responses against tumours.
Example: A mouse model expressing human PD-1 and PD-L1 allows researchers to evaluate the effectiveness of cancer vaccines in combination with anti-PD-1 or anti-PD-L1 therapies, mimicking human checkpoint interactions in the tumour microenvironment.
6. Spontaneous Tumour Models
In spontaneous tumour models, mice naturally develop tumours over time, closely mimicking human cancer development. These models allow for the study of long-term vaccine efficacy and immune surveillance in a more authentic setting.
Example: The MMTV-PyMT model for breast cancer can be used to assess vaccine responses as tumours develop spontaneously in the mammary tissue of mice.
Each of these mouse models brings unique advantages and insights into the study of cancer vaccines, helping to unravel complex interactions between vaccines, tumour cells, and the immune system.
GemPharmatech: Advanced Mouse Models and Customised Services for Vaccine Research
GemPharmatech offers a comprehensive range of genetically engineered mouse models designed to support various research applications, including cancer vaccine studies and immunotherapy research. Their portfolio includes humanised immune checkpoint mouse models, ideal for preclinical testing of immunotherapies like PD-1/PD-L1 inhibitors, as well as models with specialised modifications for oncology, metabolic disorders, and more. In addition to providing ready-to-use models, GemPharmatech offers custom genetic modification services, and preclinical services ensuring tailored solutions for specific research needs. Their expertise accelerates the discovery and development process by providing reliable, high-quality mouse models and related services.
References:
Achyut BR, Zhang H, Angara K, Mivechi NF, Arbab AS, Ko L. Oncoprotein GT198 vaccination delays tumor growth in MMTV-PyMT mice. Cancer Lett. 2020 Apr 28;476:57-66. doi: 10.1016/j.canlet.2020.02.005. Epub 2020 Feb 12.
Arbelaez, C.A., Estrada, J., Gessner, M.A. et al. A nanoparticle vaccine that targets neoantigen peptides to lymphoid tissues elicits robust antitumor T cell responses. npj Vaccines 5, 106 (2020). https://doi.org/10.1038/s41541-020-00253-9
De Robertis M, Lampreht Tratar U, Signori E, Komel T, Čemažar M. Mouse Melanoma Model in Tumor Vaccines and Immunotherapy Research. Methods Mol Biol. 2024;2773:157-163. doi:10.1007/978-1-0716-3714-2_14
Donninger H, Li C, Eaton JW, Yaddanapudi K. Cancer Vaccines: Promising Therapeutics or an Unattainable Dream. Vaccines (Basel). 2021 Jun 18;9(6):668. doi: 10.3390/vaccines9060668.
Fan, T., Zhang, M., Yang, J. et al. Therapeutic cancer vaccines: advancements, challenges and prospects. Sig Transduct Target Ther 8, 450 (2023). https://doi.org/10.1038/s41392-023-01674-3
Grimmett E, Al-Share B, Alkassab MB, Zhou RW, Desai A, Rahim MMA, Woldie I. Cancer vaccines: past, present and future; a review article. Discov Oncol. 2022 May 16;13(1):31. doi: 10.1007/s12672-022-00491-4.
Hargrave A, Mustafa AS, Hanif A, Tunio JH, Hanif SNM. Recent Advances in Cancer Immunotherapy with a Focus on FDA-Approved Vaccines and Neoantigen-Based Vaccines. Vaccines (Basel). 2023 Oct 25;11(11):1633. doi: 10.3390/vaccines11111633.
Hernandez R, Malek TR. Fueling Cancer Vaccines to Improve T Cell-Mediated Antitumor Immunity. Front Oncol. 2022;12:878377. Published 2022 May 16. doi:10.3389/fonc.2022.878377
Li X, You J, Hong L, Liu W, Guo P, Hao X. Neoantigen cancer vaccines: a new star on the horizon. Cancer Biol Med. 2023 Dec 29;21(4):274–311. doi: 10.20892/j.issn.2095-3941.2023.0395.
Singh J, Bowne WB, Snook AE. Cancer Vaccines and Immunotherapy for Tumor Prevention and Treatment. Vaccines (Basel). 2021 Nov 9;9(11):1298. doi: 10.3390/vaccines9111298.
Tay BQ, Wright Q, Ladwa R, Perry C, Leggatt G, Simpson F, Wells JW, Panizza BJ, Frazer IH, Cruz JLG. Evolution of Cancer Vaccines-Challenges, Achievements, and Future Directions. Vaccines (Basel). 2021 May 20;9(5):535. doi: 10.3390/vaccines9050535.
Vishweshwaraiah YL, Dokholyan NV. mRNA vaccines for cancer immunotherapy. Front Immunol. 2022;13:1029069. Published 2022 Dec 14. doi:10.3389/fimmu.2022.1029069