Table of Contents
Extracellular vesicles (EVs) are revolutionising our understanding of cell-to-cell communication. These ubiquitous messengers encased in a lipid bilayer membrane, are nano-sized packages actively released by virtually all cell types. Ranging from a mere 30 nanometers to a surprising 5 microns in diameter, EVs bridge the gap between cells, acting as a sophisticated communication network within the body. They shuttle a diverse cargo of biomolecules, including proteins, lipids, and nucleic acids (mRNA, miRNA), that can significantly influence the recipient cell’s phenotype and behaviour. This intricate communication system plays a critical role in numerous physiological processes, from orchestrating immune responses to facilitating tissue repair and development.
Delving into the Heterogeneity of EVs:
Despite their shared role as cellular messengers, EVs exhibit remarkable heterogeneity. They differ significantly in their biogenesis, size, and cellular origin. This diversity necessitates their classification into distinct categories. The three main classes of EVs are:
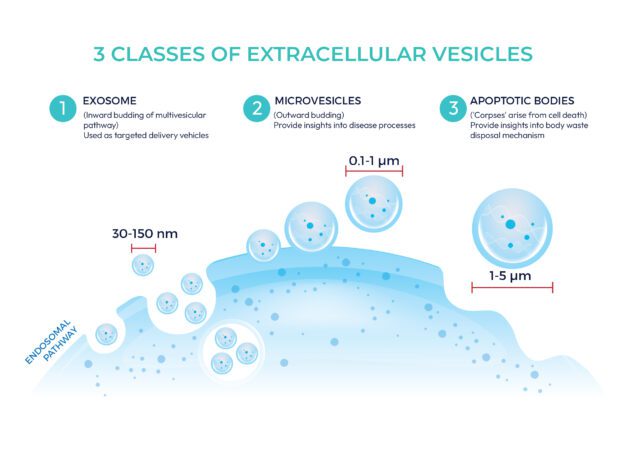
- Exosomes: The smallest EVs (30-150 nm), exosomes are formed through a meticulous intracellular journey. They originate as inward buddings of multivesicular bodies (MVBs) within the endosomal pathway. MVBs then fuse with the cell’s outer membrane, releasing exosomes into the extracellular space. Due to their defined cargo and well-understood biogenesis pathway, exosomes are particularly promising candidates for therapeutic applications. Researchers envision them as targeted delivery vehicles for therapeutic drugs or genetic material to diseased cells.
- Microvesicles: Larger than exosomes, with a diameter ranging up to 1 micrometre, microvesicles are shed directly from the cell’s plasma membrane via outward budding. This rapid-release mechanism allows cells to quickly expel cellular components in response to specific stimuli. Microvesicles hold immense potential for uncovering how cells react to stress or injury, providing valuable insights into disease processes.
- Apoptotic bodies: The largest class of EVs, ranging from 1 to 5 microns in diameter, apoptotic bodies arise from a more sombre cellular event – programmed cell death (apoptosis). During apoptosis, dying cells fragment into apoptotic bodies containing remnants of the cell’s cytoplasm and organelles. These “cellular corpses” act as signalling molecules, attracting phagocytes – immune cells that clear away cellular debris. By studying apoptotic bodies, researchers can gain insights into the body’s waste disposal mechanisms and potentially modulate them for therapeutic benefit.
Cell Sources for Therapeutic Extracellular Vesicles
The success of therapeutic EVs hinges on a critical choice: the cell source. Different cell types produce EVs with unique cargo – a cocktail of proteins, lipids, and nucleic acids – that shape the therapeutic potential of these nano-messengers. EVs can be harnessed from two main sources: body fluids and in vitro cell cultures.
EVs from Bodily Fluids:
Our bodies harbour a wealth of EVs in various fluids, including plasma, urine, and even milk. Plasma-derived EVs, with their anti-inflammatory, antioxidant, and pro-angiogenic (blood vessel promoting) properties, are being explored for treating diverse conditions, such as cardiac damage, osteoarthritis, wounds, ageing, and muscle injuries. Platelet-enriched plasma (PRP) emerges as another exciting source, offering EVs with regenerative potential. PRP-derived EVs can promote cell proliferation and blood vessel formation, aiding tissue repair after injuries.
Breast milk extends far beyond its role as a source of essential nutrients for newborns. Recent research highlights the presence of a unique population of EVs within breast milk. These human milk-derived EVs possess a remarkable repertoire of therapeutic effects, including promoting gut maturation, mitigating intestinal damage, modulating the immune system, and even attenuating viral infections. This underscores the remarkable versatility of EVs and their potential to significantly impact infant health.
By harnessing the potential of EVs isolated from bodily fluids, researchers are unlocking a novel class of therapeutic tools. These naturally occurring messengers hold immense promise for revolutionising how we approach the treatment and management of various diseases and conditions.
EVs from in vitro Cell Cultures
EVs can also be isolated from cell cultures for therapeutical purposes. Mesenchymal stem cells (MSCs) are the rockstars of this approach, particularly valued for their immunomodulatory properties. MSC-derived EVs have a knack for suppressing excessive immune responses, making them prime candidates for treating inflammatory diseases. The therapeutic efficacy of MSC-derived EVs has been investigated in many diseases, including osteoarthritis, wound healing, and liver injury.
Beyond MSCs, the world of neural cells holds promise. Studies reveal that EVs isolated from neural cells like microglia and astrocytes, or even neural stem cells, can exert neuroprotective effects. This translates to potential improvements in motor and cognitive function after traumatic brain injury.
Almost all immune cell types, including T cells, B cells, dendritic cells, macrophages, and neutrophils, have been shown to produce EVs. These immune cell-derived EVs are pivotal players in orchestrating and modulation of immune responses. These EVs typically contain the same markers as their parental cells. For instance, NK-derived EVs contain typical NK cell markers and can deliver a cargo of cytotoxic proteins, including perforin, and granzymes that destroy target cells.
By strategically selecting the cell source, researchers can harness the unique therapeutic properties of EVs. From immunomodulation to tissue regeneration, these tiny powerhouses hold immense potential for revolutionising medicine.
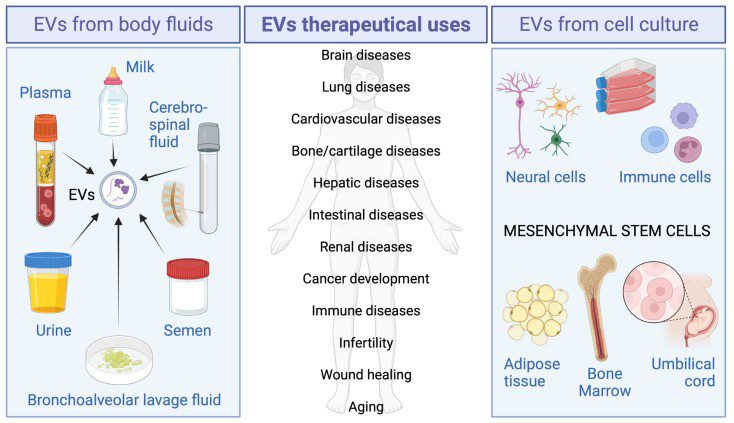
Figure 2: Therapeutic EVs sources and their possible clinical applications.
Credit: Sanz-Ros J, Mas-Bargues, C et. al., doi: 10.3390/ijms24032344
Reproduced under the Creative Commons license
7 Techniques to Isolate Extracellular Vesicles
The burgeoning field of EV research hinges on the development of robust isolation techniques. To fully elucidate the biological roles of EVs and translate their potential into clinical applications, researchers require methods that preserve their inherent heterogeneity. These methods leverage the distinct biophysical and biochemical properties of EVs, including size, density, morphology, and surface marker expression. Selecting the optimal isolation technique is paramount, as it directly influences the purity, yield, and ultimately, the functional integrity of the isolated EV population.
In pursuit of the ideal isolation strategy, researchers are continuously refining existing techniques and exploring novel approaches. The ultimate goal lies in achieving high-purity EV isolates with preserved structural and functional characteristics, allowing for a comprehensive understanding of their biological roles and therapeutic potential. Here, we will explore the most commonly utilised EV isolation methods:
Table 1: Different techniques for isolating extracellular vesicles.
Isolation Techniques | Mechanism | Advantages | Disadvantages |
Differential Centrifugation | Uses high centrifugal force to separate EVs based on size and density. | – Considered the “gold standard” – Yields intact EVs | – Time-consuming – Prone to operator error – May damage EVs – Low purity (requires additional steps) |
Density Gradient Centrifugation | Similar to differential centrifugation, but separates EVs based on their buoyant density in a gradient medium. | – Higher purity than differential centrifugation – Separates EVs by subpopulations | – More complex and expensive – Requires specialised equipment |
Ultrafiltration | Uses membranes with different pore sizes to separate EVs based on size. | – Relatively simple and fast – Can be automated | – May clog membranes – Lower purity – May damage EVs |
Polymer-based Precipitation | Uses polymers to precipitate EVs out of solution. | – Fast and inexpensive – Can be used with small sample volumes | – Lower purity – May not be suitable for all EV types |
Size Exclusion Chromatography (SEC) | Separates EVs based on their size as they flow through a column packed with beads. | – Gentle on EVs – Good for separating EVs from protein contaminants | – Lower yield compared to centrifugation methods – May not separate all EV subpopulations |
Immunoaffinity Isolation | Uses antibodies specific to EV surface markers to capture EVs. | – High purity – Isolates specific subpopulations of EVs | – Expensive – Requires knowledge of specific EV surface markers |
Microfluidic Devices | Utilises microchannels to manipulate and isolate EVs based on size, density, or electrical properties. | – Precise control over EV isolation – Potential for automation – High potential for future development | – Relatively new technology – Limited availability of commercial devices – May require specialised expertise |
*Checkout our qEV SEC columns for simple and rapid EVs isolations!
3 Techniques for Physical Analysis of Extracellular Vesicles
Physical analysis of exosomes is crucial in the field of exosome research, particularly when exploring their therapeutic potential. Physical analysis helps determine the size, density, surface molecules, and purity which is essential for understanding how exosomes behave in the body and their potential for targeted delivery. This also provides a standardized way to characterise exosomes, allowing researchers to compare results across different studies and laboratories, which is vital for ensuring the reproducibility and reliability of therapeutic approaches.
Nanoparticle Tracking Analysis (NTA): This technique allows for the determination of exosome size and concentration. It combines the properties of both laser light scattering microscopy and Brownian motion to obtain size distributions of particles in liquid suspension. The machine is normally equipped with one or more lasers and an optical microscope connected to a digital camera. Particles are visualised by the light they scatter upon laser illumination, and the Brownian motion of individual exosomes is monitored. The software analyses the video frame-by-frame, tracking the movement of each exosome. By applying the Stokes-Einstein equation, which relates particle size to its Brownian motion, the software calculates the hydrodynamic diameter of each exosome. The software generates a size distribution profile, revealing the number of exosomes at various size ranges. Additionally, by analysing the total number of tracked particles within a defined volume, NTA can estimate the exosome concentration in the sample.
Dynamic Light Scattering (DLS): Like NTA, dynamic light scattering, or DLS, uses the scattered light due to the Brownian motion of particles to estimate particle size and concentration. However, DLS measures the fluctuations in the intensity of scattered light caused by the Brownian motion of particles in a solution. By analysing the pattern of fluctuations in the scattered light intensity, DLS can determine the size distribution of the particles in the solution, including the size range of exosomes present. The data obtained from DLS analysis are typically presented as a size distribution plot, which shows the relative abundance of exosomes at different size ranges within the sample. While DLS is a powerful technique for analysing the size distribution of exosomes, it only provides estimation to the average hydrodynamic diameter of the entire particle population in the sample. Unlike NTA, DLS doesn’t provide information on individual exosome sizes.
Tunable Resistive Pulse Sensing (TRPS): This emerging technique offers a high-resolution analysis of exosome size, concentration and zeta potential as they pass through a size-tunable nanopore. TRPS measures the change in electrical current as individual exosomes pass through the nanopore. As individual exosomes pass through the pore, they briefly block some of the currents. TRPS measures these current blockades. The magnitude and duration of the current blockade are related to the size of the particle that caused it. By analysing these blockades, TRPS can provide information on individual exosome sizes. The resolution capabilities of TRPS are much higher than NTA as NTA struggles to properly resolve subpopulations of multimodal samples and has been shown to overestimate particle concentration.
*Checkout The Exoid, the latest instrument for TRPS analysis!
Table 2: Comparison of different techniques for physical analysis of EVs.
Tunable Resistive Pulse Sensing (TRPS) | NTA (Nanoparticle Tracking Analysis) | Dynamic Light Scattering (DLS) |
Single-particle technique | Single-particle technique | Ensemble technique |
Particle size is directly proportional to blockade magnitude; each particle is measured individually | Brownian motion is tracked for individual particles and the resulting diffusion coefficient is used to calculate the hydrodynamic diameter | Analysis of the fluctuations in scattered light intensity caused by Brownian motion of particles in solution |
Using appropriately sized nanopores, particles can be measured across a wide size range (40 nm–11 µm) | Smaller size range (approximately 10 – 2000 nm) | Wider size range (1 nm –10 µm) – but strong limitations for multimodal samples |
Automated data processing with user-friendly data visualisation interface | Advanced data analysis required | Simple protocols |
Can resolve populations in multimodal samples | Resolution of multimodal samples is relatively limited | Cannot resolve multimodal samples |
Not dependent on optical properties of particles and dispersant | Accurate knowledge of the optical properties of particles and dispersants is required | Accurate knowledge of the optical properties of particles and dispersants is required |
Each technique has its own strengths and limitations in exosome analysis. NTA is known for its high resolution and sensitivity, DLS offers rapid analysis suitable for high-throughput applications, and TRPS provides absolute size measurements with high resolution. The choice of technique depends on the specific requirements of the study and the characteristics of the exosome sample being analysed.
Extracellular Vesicles in Regenerative Medicine
Given their rich content of biomolecules like lipids, proteins, and nucleic acids (including microRNA, messenger RNA, and DNA), along with small molecules inherited from parent cells, EVs play pivotal roles in physiological and pathological processes such as cell proliferation, immune modulation, angiogenesis, and tissue repair. Consequently, they are increasingly recognised as promising nanotherapeutics in regenerative medicine.
Indeed, EVs offer substantial advantages over conventional stem cell therapies in regenerative medicine, including multiple therapeutic effects, efficient cargo delivery, enhanced biocompatibility and safety, as well as improved reproducibility and stability. The therapeutic mechanisms of native EVs primarily involve cell-surface interactions with target cells and subsequent cargo transfer, activating signalling pathways that mitigate disease effects and promote regenerative functions.
Beyond native EVs, the field is actively exploring the potential of engineered EVs. These customised EVs offer a new frontier in targeted therapy. By incorporating specific therapeutic agents into their cargo, engineered EVs can act as targeted delivery vehicles. This approach unlocks the potential for a more potent and progressive regenerative effect compared to native EVs alone.
The future of regenerative medicine holds immense promise with EVs as the cornerstone. Research is actively investigating how EVs can be harnessed to promote healing and restore function in a wide range of organs, including the brain, heart, skin, bones and cartilage, liver, and kidneys. As research progresses, EVs have the potential to revolutionise the way we approach tissue regeneration and restore health.
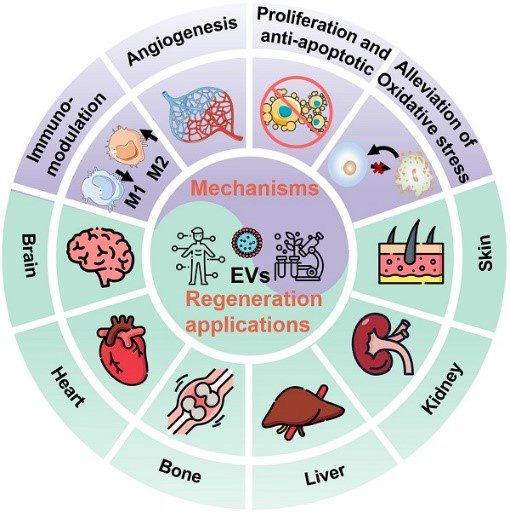
Figure 3: Schematic illustration of the EVs therapeutic mechanisms and regeneration applications in various organs including brain, heart, bone, liver, kidney, and skin.
Credit: Li M., Fang F., et. al., doi: 10.7150/thno.72812
Reproduced under the Creative Commons license
References:
Chutipongtanate, S.; Morrow, A.L.; Newburg, D.S. Human Milk Extracellular Vesicles: A Biological System with Clinical Implications. Cells 2022, 11, 2345. https://doi.org/10.3390/cells11152345
De Bari C, Roelofs AJ. Stem cell-based therapeutic strategies for cartilage defects and osteoarthritis. Curr Opin Pharmacol. 2018;40:74-80. doi:10.1016/j.coph.2018.03.009
Doyle LM, Wang MZ. Overview of Extracellular Vesicles, Their Origin, Composition, Purpose, and Methods for Exosome Isolation and Analysis. Cells. 2019 Jul 15;8(7):727. doi: 10.3390/cells8070727.
Du, S., Guan, Y., Xie, A. et al. Extracellular vesicles: a rising star for therapeutics and drug delivery. J Nanobiotechnol 21, 231 (2023). https://doi.org/10.1186/s12951-023-01973-5
Hering C, Shetty AK. Extracellular Vesicles Derived From Neural Stem Cells, Astrocytes, and Microglia as Therapeutics for Easing TBI-Induced Brain Dysfunction. Stem Cells Transl Med. 2023 Mar 17;12(3):140-153. doi: 10.1093/stcltm/szad004.
Hu L, Wang J, Zhou X, Xiong Z, Zhao J, Yu R, Huang F, Zhang H, Chen L. Exosomes derived from human adipose mensenchymal stem cells accelerates cutaneous wound healing via optimizing the characteristics of fibroblasts. Sci Rep. 2016 Sep 12;6:32993. doi: 10.1038/srep32993. Erratum in: Sci Rep. 2020 Apr 16;10(1):6693.
Iannotta D, Yang M, Celia C, Di Marzio L, Wolfram J. Extracellular vesicle therapeutics from plasma and adipose tissue. Nano Today. 2021 Aug;39:101159. doi: 10.1016/j.nantod.2021.101159. Epub 2021 Apr 27.
Jiang W, Tan Y, Cai M, Zhao T, Mao F, Zhang X, Xu W, Yan Z, Qian H, Yan Y. Human Umbilical Cord MSC-Derived Exosomes Suppress the Development of CCl4-Induced Liver Injury through Antioxidant Effect. Stem Cells Int. 2018 Mar 4;2018:6079642. doi: 10.1155/2018/6079642.
Kumar, M.A., Baba, S.K., Sadida, H.Q. et al. Extracellular vesicles as tools and targets in therapy for diseases. Sig Transduct Target Ther 9, 27 (2024). https://doi.org/10.1038/s41392-024-01735-1
Li M, Fang F, Sun M, Zhang Y, Hu M, Zhang J. Extracellular vesicles as bioactive nanotherapeutics: An emerging paradigm for regenerative medicine. Theranostics. 2022;12(11):4879-4903. Published 2022 Jun 21. doi:10.7150/thno.72812
Pedrioli, G.; Piovesana, E.; Vacchi, E.; Balbi, C. Extracellular Vesicles as Promising Carriers in Drug Delivery: Considerations from a Cell Biologist’s Perspective. Biology 2021, 10, 376. https://doi.org/10.3390/biology10050376
Wu J, Piao Y, Liu Q, Yang X. Platelet-rich plasma-derived extracellular vesicles: A superior alternative in regenerative medicine? Cell Prolif. 2021 Dec;54(12):e13123. doi: 10.1111/cpr.13123. Epub 2021 Oct 5.
Yang P, Peng Y, Feng Y, Xu Z, Feng P, Cao J, Chen Y, Chen X, Cao X, Yang Y, Jie J. Immune Cell-Derived Extracellular Vesicles – New Strategies in Cancer Immunotherapy. Front Immunol. 2021 Dec 8;12:771551. doi: 10.3389/fimmu.2021.771551.
Sanz-Ros J, Mas-Bargues C, Romero-GarcÃa N, Huete-Acevedo J, Dromant M, Borrás C. Extracellular Vesicles as Therapeutic Resources in the Clinical Environment. Int J Mol Sci. 2023 Jan 25;24(3):2344. doi: 10.3390/ijms24032344.